Biomechanics of the cornea in keratoconus and other corneal ectasias
Purpose: The relationship between structure and function of the cornea is discussed. Altered biomechanical properties of the corneal stroma are responsible for the development of primary and secondary ectasia.
Material and Methods: A systematic literature review of the biochemical components of the corneal stroma and their importance for the biomechanics of the cornea was performed. The physical basis of the theory of elasticity and necessary extensions in terms of corneal nonlinearity and inhomogeneity are discussed. The different biomechanical conditions leading to primary ectasia (e.g. keratoconus) and secondary ectasia after Lasik surgery are compared.
Results: Primary ectasias are the result of altered biochemical properties of the stromal collagens. A different arrangement of collagen fibres in the stroma is another possible cause of primary ectasia. Post-Lasik ectasia is caused by the decoupling of the flap and the residual stroma. The wall tension within the residual posterior stroma increases by a factor after Lasik. The thinned posterior stroma is not able to permanently withstand the intraocular pressure.
Conclusion: The collagens of the stroma are decisive for the structure and thus also for the function of the cornea as an imaging element. Measurement results on the biomechanics of the cornea obtained with air-puff procedures provide only limited information on the biomechanics of the cornea and on the understanding of the aetiology of keratoconus, since here the mechanical loads to deform the cornea only act only for a few milliseconds, while the deforming force of the intraocular pressure acts permanently.
Introduction
Corneal ectasias, which include keratoconus, pellucid marginal degeneration (PMD), keratoglobus and Post-Lasik ectasias, are characterised by progressive changes in corneal curvature and decrease in corneal thickness. An indication of keratoconus is already provided by the retinoscopy. Today, the exact diagnosis of these abnormalities is made using corneal topography. Modern procedures such as Scheimpflug technology, air-puff procedures (e.g. Ocular Response Analyzer, Corvis) or optical coherence tomography (OCT) detect morphological changes in the cornea at an early stage. However, these are only secondary signs, preceded by abnormalities in the biomechanics of the cornea and especially the corneal stroma.1,2 The various newly introduced indices for describing corneal biomechanics and diagnosing keratoconus do not contribute to understanding the causes of ectatic corneal disease. The air-puff procedures exert mechanical stress on the cornea only in very short periods of a few milliseconds. In fact, however, the ectasias are the result of permanent mechanical stress due to intraocular pressure. From the point of view of biomechanics, the challenge to the cornea is threefold: on the one hand, the cornea must be soft enough to be able to permanently assume an aspheric shape under the influence of intraocular pressure; on the other hand, it must also be resistant enough so that, in order to ensure constant refraction, it is not deformed in the short term either by intraocular pressure or by shear and pressure forces of the eyelids.3,4 Finally, the third structural requirement is the transparency of the cornea. The biomechanical properties of the stroma are the prerequisite for the cornea to meet the above challenges.5 The structure therefore determines the function. Changes in the structure of the stroma, but not the epithelium, and associated abnormal biomechanical properties of the stroma are considered to be the cause of ectatic anomalies of the cornea.2
Composition and structure of the cornea
The cornea has a layered structure. The three layers of the cornea are epithelium with basement membrane, stroma with Bowman's membrane and endothelium with Descemet's membrane. The contributions of the epithelium including the basement membrane as well as Descemet's membrane and endothelium to the mechanical strength of the cornea are to be assessed as low simply because of their small thickness compared to the stroma. Furthermore, neither the epithelium nor the endothelium contain mechanically resilient structural proteins (e.g. collagen). The stroma therefore contributes most to the mechanical stability of the cornea.5 The thicker the cornea, the higher its modulus of elasticity.6 The anterior stroma, with its cross-links between collagens and higher keratocyte density compared to the posterior stroma, shows 50% higher tensile strength than the posterior stroma.7 The biochemical properties of the major components of the stroma as well as their arrangement in the stroma are the basis for the biomechanics of the cornea. The corneal stroma is mainly composed of water (78%), collagens (15%) and proteoglycans (7%). While the collagens are characterised by a high tensile strength, the proteoglycans are essential for the compressive strength and viscoelasticity of the stroma.
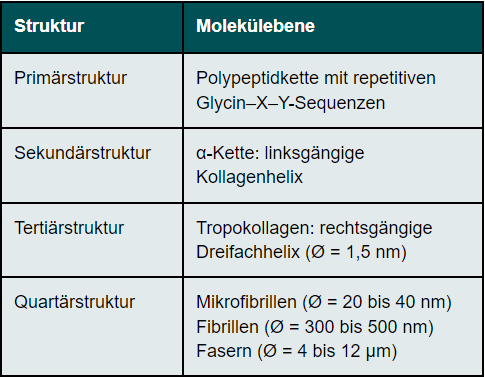
Collagens
Collagens are found in the body wherever tensile stresses act on tissues (e.g. skin, tendons, bone matrix). The tensile forces acting on the corneal stroma are wall tension stresses caused by intraocular pressure. The four straight eye muscles also exert tensile forces on the collagens. Collagens are formed by the keratocytes of the corneal stroma. Based on studies of corneal transplants, a turnover rate of collagens of 2 to 3 years could be determined.8 Type I fibrillar collagens are present in the corneal stroma. (Figure 1)
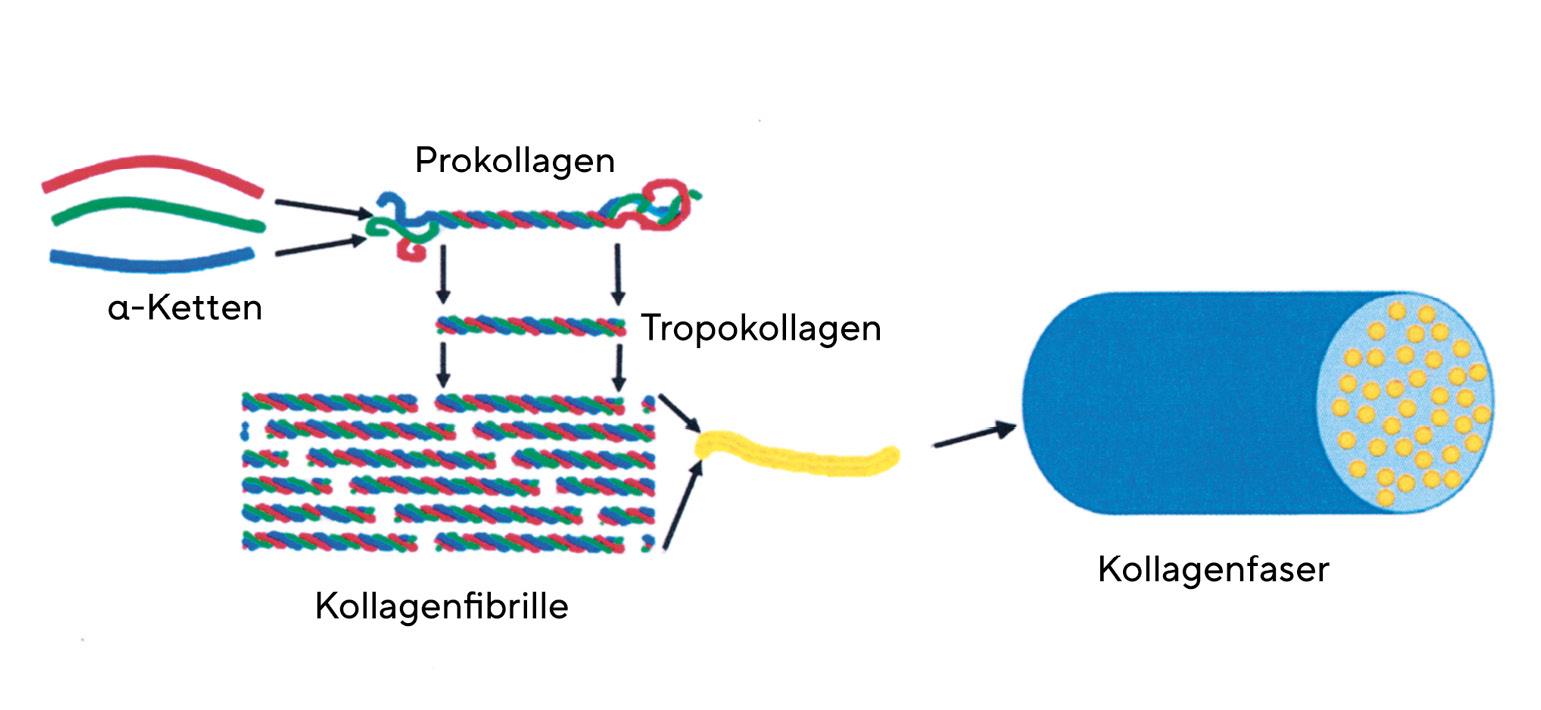
Structure of collagens
Collagens have a complex hierarchical structure, which results in their high tensile strength. The primary structure of collagens has a high degree of regularity. Every third amino acid of the collagen molecule is glycine, so that the molecule is built up of repetitive sequences consisting of glycine - X - Y. The amino acids X and Y are very often represented by the amino acids proline (X) and 4-hydroxyproline (Y). This basic structure of collagen comprises between 200 and 1000 amino acids. (Table 1)The secondary structure of collagen is given by the left-hand α-chain. Three α-chains are twisted around each other. They thus form a right-handed triple helix called tropocollagen (tertiary structure). The diameter of the tropocollagen is 1.5 nm. The α-helices are cross-linked to each other via the amino acid hydroxylysine. The close twisting of the three individual chains into a triple helix is the reason for the high tensile strength of the collagen. The quaternary structure of collagen is determined by the assembly of several tropocollagens. Triple helices first form the microfibrils, which in turn assemble into collagen fibrils. Several collagen fibrils join together to form collagen fibres. There are also cross-links between the collagen fibrils, the number of which increases with age, making the cornea more brittle. The cross-links prevent the collagen fibrils from sliding past each other in an uncontrolled manner. The cornea's resistance to deformation increases with the degree of cross-linking.9 The collagen fibres are in turn arranged in lamellae. The size of the lamellae is variable. In the centre of the cornea, 200 lamellae are arranged one above the other, so that they make up the entire thickness of the corneal stroma. The packing density is higher in the anterior stroma than in the posterior stroma.10 The collagen has rigid and elastic domains. In the single chain, there are regions that contain neither proline nor hydroxyproline. These domains are elastic, while those rich in proline and hydroxyproline are rigid. They stabilise the collagen molecule against mechanically induced stress. Collagens can stretch as triple helices slide past each other. An increase in length of the molecule is also possible by stretching the elastic domains.
Arrangement of the collagen fibrils
The orientation of the collagen fibrils is decisive for both the transparency of the cornea and its direction-dependent mechanical properties. In the human cornea, the collagen fibrils are arranged almost orthogonally to each other in the central cornea. This arrangement is maintained up to a distance of 2 mm from the limbus, where the collagens change into a ring-shaped or tangential arrangement. The limbus is subjected to a strong mechanical stress, as here there is an abrupt transition from a steep curvature of the cornea with a radius of curvature of up to 9 mm to a flatter curvature of the sclera with a radius of curvature of about 13 mm.11 The annular arrangement of the collagen fibrils is a prerequisite for ensuring that the precise optics of the central cornea are not lost due to geometric discontinuity at the limbus. The orthogonal arrangement of the collagen fibrils forms a diffraction grating that is decisive for transparency. In addition, since the collagen fibrils can be seen as extensions of the tendons of the four straight eye muscles, it provides maximum stability of the corneal structure. That eye movements determine the arrangement of the collagen fibrils is evident from the fact that some species, such as cattle or horses, have an almost exclusively vertical orientation of the collagen fibrils, since in them only the upper and lower straight eye muscles are predominantly responsible for stabilising the retinal image.12
Anterior stroma and mechanical resilience of the cornea
The anterior stroma, with a thickness of 100 to 120 µm, differs significantly from the posterior stroma in terms of the structure and arrangement of collagens. With a thickness of 0.2 to 1.2 µm, the collagen lamellae of the anterior stroma are significantly thinner and, with a maximum width of 30 µm, also considerably narrower than the lamellae of the posterior stroma. Unlike a large part of the lamellae of the posterior stroma, the lamellae do not have a preferred direction; they run obliquely with random orientation through the stroma.10 A large number of collagen lamellae originate at the limbus and terminate in Bowman's membrane. Unlike the collagen lamellae of the posterior stroma, the lamellae of the anterior stroma can split horizontally and/or vertically. Thus, two to three partial lamellae can arise from one original lamella, which in turn can unite with partial lamellae of other layers of the anterior stroma. For this reason, the collagen lamellae of the anterior stroma cannot be clearly distinguished from each other. This increases the mechanical resistance of the anterior stroma. The anterior stroma is therefore considered the structure that gives the cornea a constant shape. In keratoconus, the collagen fibrils are arranged differently.13 The more irregular structure of the anterior stroma compared to the posterior stroma causes an increase in light scattering of the anterior stroma by a factor of 2.8
Proteoglycans
Proteoglycans are macromolecules consisting of a core protein to which glycosaminoglycans are covalently bound as side chains. They play an essential role in building up the extracellular matrix in the corneal stroma. The arrangement and course of the collagen fibres in the corneal stroma is determined by the proteoglycans. The two most important glycosaminoglycans of the corneal stroma are keratan sulphate and dermatan sulphate. The proportion of keratan sulphate in the stroma depends on the amount of oxygen available. Obviously, a low oxygen concentration promotes the formation of keratan sulphate. In organisms with a thick cornea, keratan sulphate dominates in the posterior stroma because the oxygen concentration is lower here than in the anterior stroma.14,15 Keratan sulphate regulates the diameter of the collagen fibrils, dermatan sulphate determines the spacing of the collagen fibrils and ensures adhesion between the collagen lamellae.16,17 The proteoglycans act on the collagens via electrostatic forces.15 A deficiency or defect of keratan sulphate has been observed in keratoconus as well as pellucid marginal degeneration.18 Glycosaminoglycans are negatively charged electrically due to their sulphate groups (SO3-) and carboxyl groups (COO-). These electrical charges are essential for the biomechanics of the cornea in two ways. Water binds to these charges due to its dipole character, which determines the water content and thus the swelling state of the cornea. The water content is not only important for the transparency of the cornea, the elasticity modulus of the stroma also depends on the swelling state of the cornea. The higher the water content of the stroma, the lower its modulus of elasticity.19 A dehydrated and therefore thinner cornea, on the other hand, has a higher modulus.6 The repulsive forces of the negative charges among themselves determine the volume of the stroma. If all the negative charges of the glycosaminoglycans were neutralised by positive ions (e.g. Na+), the volume of the stroma would shrink to a quarter of its original value.
Mechanical properties of the cornea
The cornea is a mechanotransducer, i.e. an external mechanical stress (e.g. intraocular pressure, shearing forces of the blink, contact lenses) causes a measurable change in the structure of the cornea. Energy dissipation prevents mechanical stress from damaging the cornea. Like many tissues in the organism, the cornea shows viscoelastic behaviour. The elastic behaviour of the cornea is linked to the tensile strength of the collagens, while the distribution of water in the stroma and the electrostatic interactions between collagens and proteoglycans determine the time-dependent viscous properties of the cornea.
Elasticity
The elasticity of the cornea is based on the reversible stretching of the elastic domains of the collagen. A linear elastic material can be described by Hooke's law, which states a simple relationship between the external stress σ and the deformation ε of the material. Accordingly, the following equation applies σ = E ε. A linear elastic material is characterised by a constant modulus of elasticity E. The cornea is not a linear elastic material, i.e. its modulus of elasticity itself depends on the deformation. However, since non-linear materials also exhibit almost linear behaviour at low external stresses, a constant modulus of elasticity can be approximately specified for the cornea. (Figure 2) Since the cornea is neither an isotropic nor a homogeneous material, its biomechanical properties are dependent on position. The mean elastic modulus of the central cornea is 4.2 ± 1.2 MPa anteriorly and 2.3 ± 0.7 MPa posteriorly.20 (Figure 3)
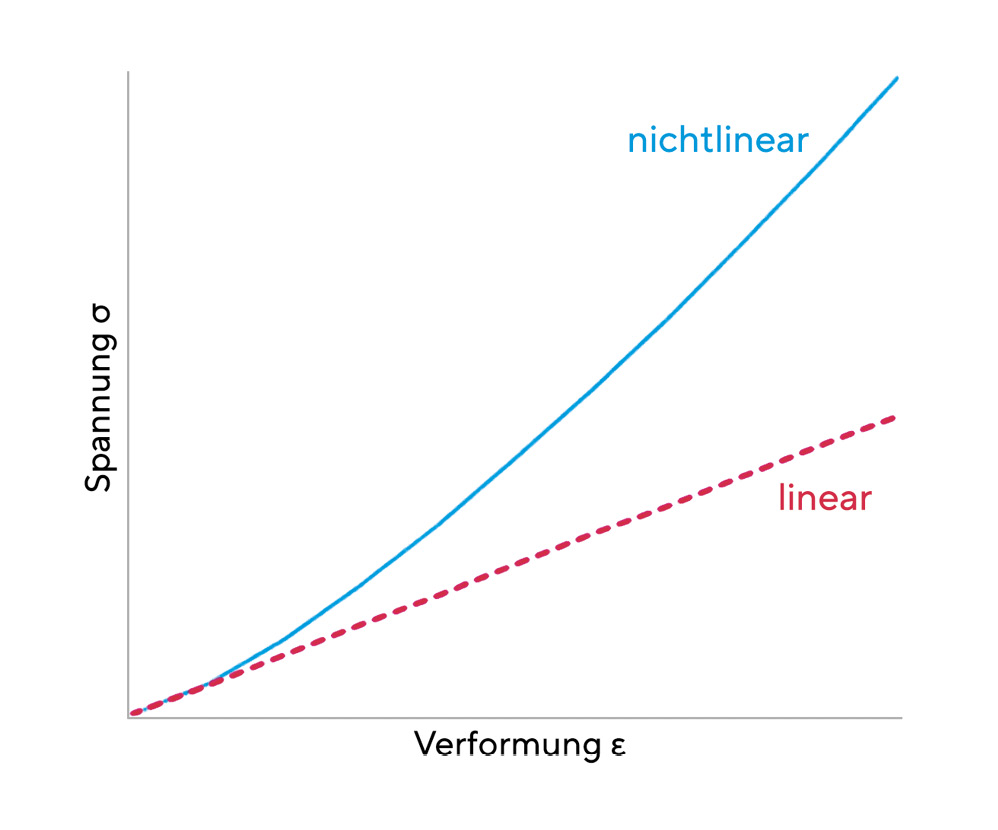
Viscoelasticity
The cornea is viscoelastic. A viscoelastic material is characterised by a different temporal behaviour of deformation and relaxation. A viscoelastic deformation is fully reversible, but on a different time scale than an elastic material. An impressive case of corneal viscoelasticity is described by Marta et al.21 They report a case of transient keratoconus following a car accident with airbag trauma. The cornea was mechanically deformed by the impact of the airbag, which lasted only fractions of a second, to such an extent that the patient developed the symptomatology of keratoconus. Due to the shape memory of the cornea, which is based on the viscoelasticity of the cornea, the cornea returned to its original shape within a period of nine months. The different behaviour of viscoelastic materials and elastic materials is due to changes in the structure in the molecular range. Viscoelasticity is the result of a time-dependent dissipation of energy. In thermodynamics, dissipation refers to an irreversible process in which energy (e.g. kinetic energy, potential energy) is converted into another form such as heat. On a molecular level, it can be explained by the sliding of the collagen fibrils past each other and of the collagen lamellae within the proteoglycan matrix. This sliding transfers energy in the form of heat to the stoma (mechanothermal energy transfer).
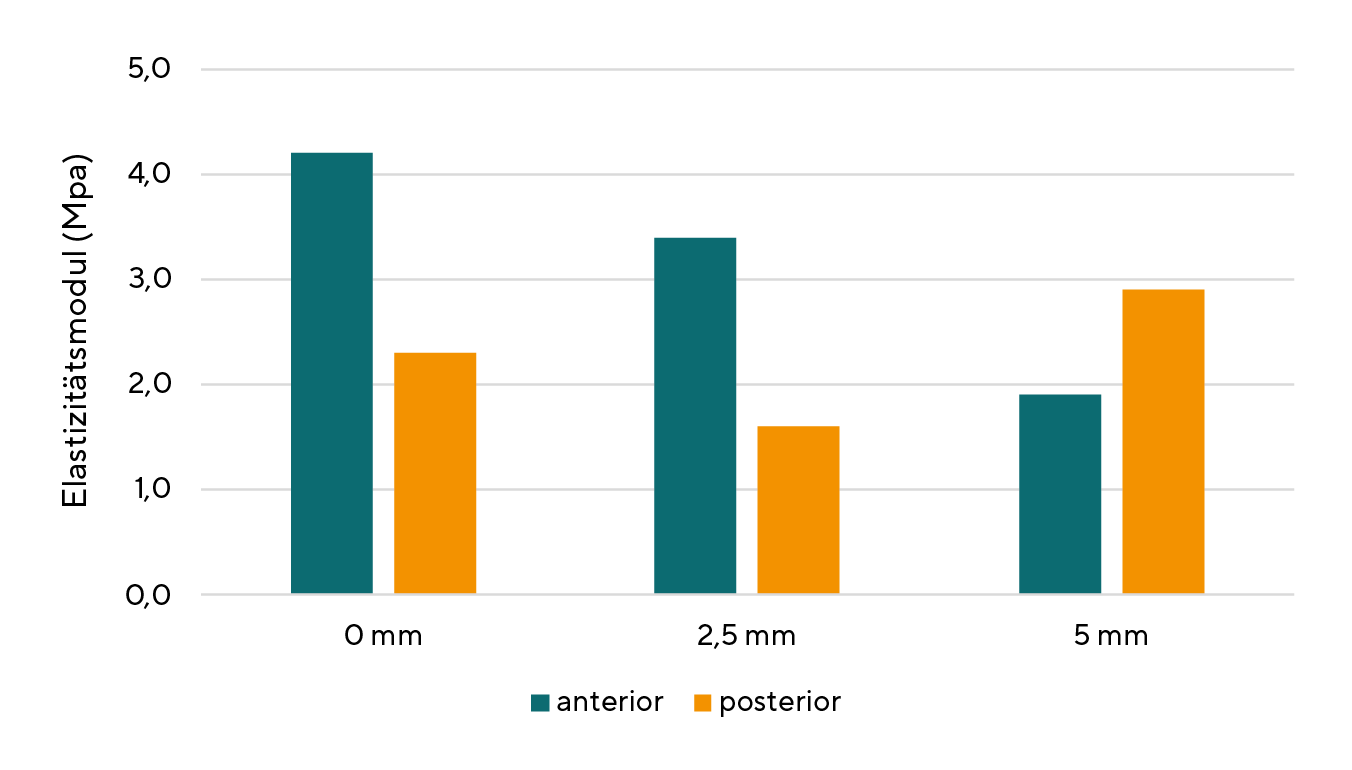
Corneal ectasias (keratectasias)
Corneal ectasias are a group of non-inflammatory diseases and abnormalities of the cornea characterised by thinning and altered curvatures of the cornea. Keratoconus and pellucid marginal degeneration are the most common primary keratectasias. Post-Lasik ectasia is a secondary keratectasia as a complication of Lasik surgery.
Primary keratectasias
Keratoconus
Keratoconus is the most common primary ectasia. Its prevalence in Caucasians ranges from 10 to 90 cases per 100,00 people. In the Middle East and India, keratoconus is much more common than in other areas of the world, with a prevalence of up to 2300 cases per 100,000 people.22 The incidence of keratoconus is significantly lower in Caucasians, with rates of 1.5 to 4.5 cases per 100,000 persons per year than in Asians, with incidence rates of 19 to 25 new diseases per 100,000 persons per year.22 The incidence of keratoconus is also determined by the diagnostic procedures used. The prevalence of keratoconus has increased with the use of imaging and topographic techniques that allow diagnosis at a very early stage of disease. Keratoconus is a degenerative, non-inflammatory and often progressive disease of the cornea. The characteristic feature of keratoconus is a severe protrusion of the cornea, which typically occurs below the centre of the cornea. The cornea may be thinned. Myopia and an increasingly irregular astigmatism are the consequences of the bulging. Scarring may occur in the area of Bowman's membrane. Stretch marks (striae) can be observed in the deeper stroma. Around the cone, iron deposits known as Fleischers' ring may occur in the deeper epithelium. (Figure 4)
Pellucid marginal degeneration
PMD is a degenerative disease of the cornea that is often confused with keratoconus. PMD differs from keratoconus in three ways. The onset of the disease is later than in keratoconus, at an age of 20 to 30 years. The changes occur further in the periphery, so that central vision is hardly affected at first. Unlike keratoconus, PMD does not stop but progresses slowly. PMD is characterised by bilateral thinning in the lower periphery of the cornea. The thinned area has a crescent or arcuate geometry. Above the thinned area, the corneal thickness is within the normal range; however, it may be bulged out anteriorly, causing a higher irregular astigmatism. In the keratograph image, the altered topography of the cornea is regularly visible as a "kissing bird".
Keratoglobus
A rare primary keratectasia is keratoglobus, which can occur in two forms, congenital or as a variant acquired in adulthood. The acquired form can be the final stage of keratoconus. The thinning of the cornea in keratoglobus extends from limbus to limbus, with the thinning being most pronounced in the outer and middle periphery. The cornea is bulged out in a spherical shape. Due to the extensive thinning, perforations of the cornea can easily occur. In congenital keratoglobus, Bowman's membrane is absent. Descemet's membrane is thickened but has numerous tears.23
Terrien's marginal degeneration
The clinical sign of Terrien's marginal degeneration is a slowly progressive, bilateral thinning of the peripheral cornea. In most cases, the upper corneal periphery is affected. As the disease progresses, the thinning may extend to the entire corneal periphery. Bowman's membrane is usually absent. High irregular astigmatism as a result of the severe peripheral flattening in the vertical meridian is a common complication of this corneal degeneration.24
Secondary keratectasias
Secondary keratectasias are the result of corneal surgery, and almost all known secondary keratectasias are due to laser refractive surgery on the cornea. The Lasik procedure in particular is considered the most important cause of secondary keratectasia, for which the term post-Lasik ectasia has now become common. The incidence of ectasia after Lasik surgery is reported to be between 0.04 and 0.6%.25 Its prevalence is thus higher than the prevalence of keratoconus. Most post-Lasik ectasias occur in the first few months after treatment; however, it cannot be ruled out that ectasia is also possible years later. Figure 5 shows a cornea in which keratoconus has developed 5 years after Lasik surgery.
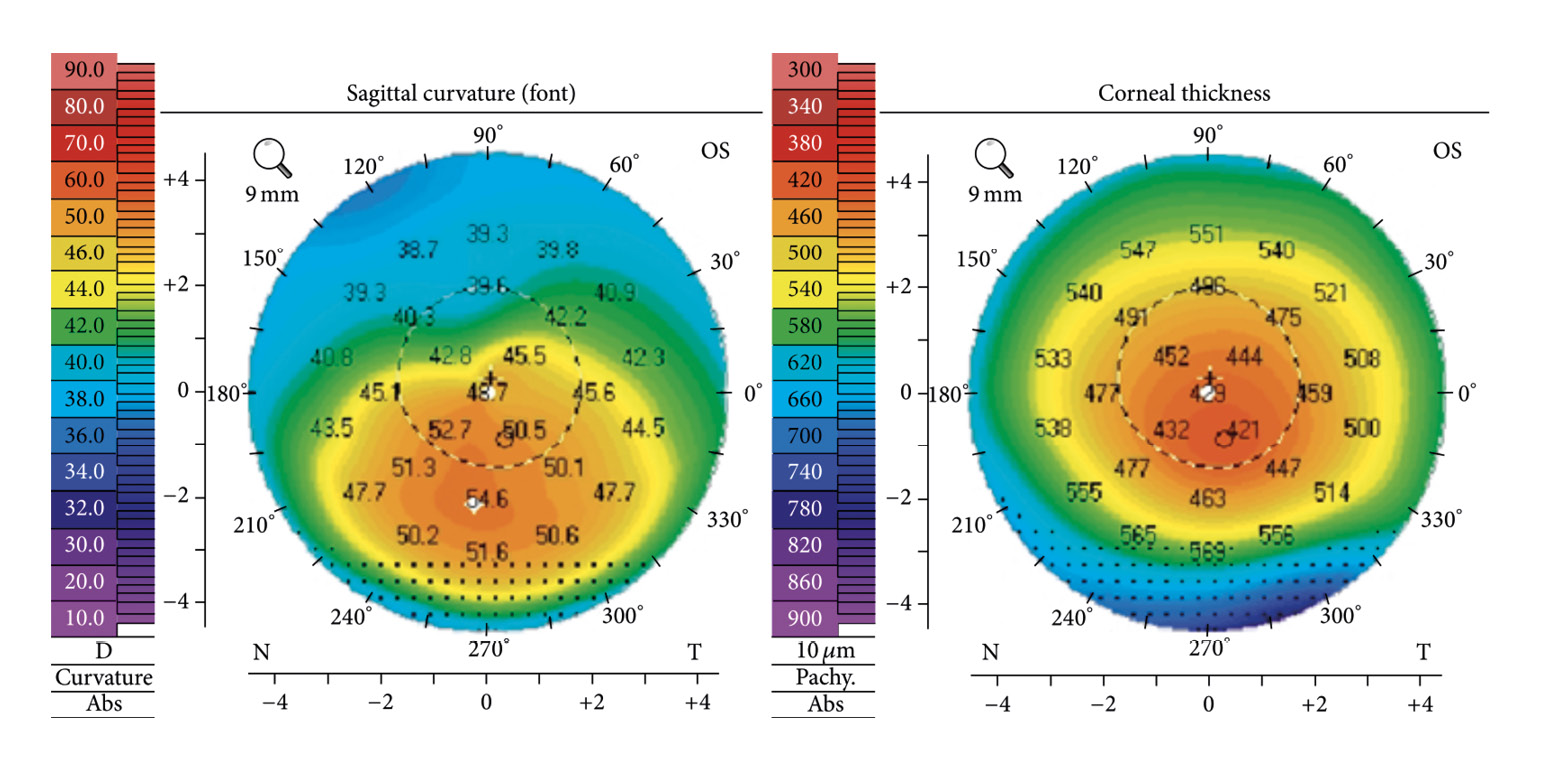
Corneal biomechanics and ectasia
The structure and stability of the cornea are determined by the biomechanical properties of the collagen and the arrangement of the collagen fibres in the stroma. As early as 1981, Andreassen et al. suggested that it is biomechanical factors that lead to the development of keratoconus.26 In addition to the collagen molecules themselves, their arrangement in the stroma of the cornea also plays an important role.
Primary ectasias
The altered biomechanical properties of the stroma can be attributed to changes in its structural components (collagens, proteoglycans). In both keratoconus and PMD, decreased amounts of keratan sulphate, which determines the diameter of collagen fibrils, have been detected.18 Collagens are responsible for the mechanical resilience of the stroma. A lack of collagens therefore weakens the cornea. Elevated concentrations of collagenases have been detected in corneas with keratoconus.27 Collagenase-3 (matrix metalloprotease 13) and other tissue-modelling proteases are highly expressed in keratoconus corneas, suggesting intracellular and extracellular degradation of collagens.28 This may be responsible for the thinning of the stroma. In addition to an increased concentration of collagenolytic matrix metalloproteases, a deficiency of TIMP-1 (tissue inhibitor of metalloporteinases), which inhibits the activity of matrix metalloproteases and thus the degradation of collagen, has also been demonstrated in keratoconus.29,30 Increased levels of tissue-modulating matrix metalloproteinases involved in collagen degradation have been detected in the tear film of patients with keratoconus and PMD.31
The resilience of collagens is also due to the fact that the helices of collagen and collagen fibrils are prevented from sliding past each other by cross-links between them. Critchfield and co-workers found a lack of hydroxylysine, which in turn leads to fewer cross-links between the helices.32 In addition to biochemical factors that weaken the tensile strength of the collagens, the arrangement and orientation of the collagen lamellae also determine the biomechanics of the cornea. Modern experimental methods such as X-ray, synchrotron radiation or Brillouin scattering33 allow a deeper insight into the structure of the stroma. The high mechanical resilience of the anterior stroma is due to cross-links between the collagen lamellae. In corneas with keratoconus, these cross-links are significantly reduced. There is also a lack of collagen lamellae that insert into Bowman's membrane.34 X-ray diffraction has shown that the orthogonal arrangement of collagens in the central cornea as a continuation of the main direction of pull of the four straight external eye muscles is no longer present in keratoconus. Instead of an orientation of the collagens in superior-inferior (90° - 270°) and nasal-temporal (0°-180°) directions, a preferential orientation in 60° and 120° is shown.35 With synchrotron radiation, an irregular arrangement of the collagens could be detected, especially in the area of the corneal apex.13 The inter- and intralamellar displacement of the collagen fibres also leads to low cohesive forces between the collagens and thus promotes a mutual sliding past of the collagens. Higher forces occur in the region of the bifurcation of the collagen lamellae. According to Meek, the irregular arrangement and sliding of the collagens lead to thinning of the cornea and the associated steepening of the corneal curvature.13 Another approach to explaining the development of keratoconus assumes a localised, i.e. focal, origin rather than a generalised weakness of the stroma.2,36 The starting point of the processes leading to keratoconus is a locally limited reduced elastic modulus. Mechanically weak areas of the cornea are characterised by a low elastic modulus. (2) Changes in corneal geometry also affect the distribution of mechanical stress in the cornea. Thin and flat areas of the cornea are more exposed to stress than thick and steep corneal areas, according to Roberts.2 If these areas are surrounded by tissue with a higher modulus of elasticity, the weaker area will thin out more under the influence of intraocular pressure than the more mechanically resistant areas. The thinning of the cornea further increases the asymmetry of the distribution of stress in the cornea. The cornea will steepen to compensate for the local stress. (Figure 6)
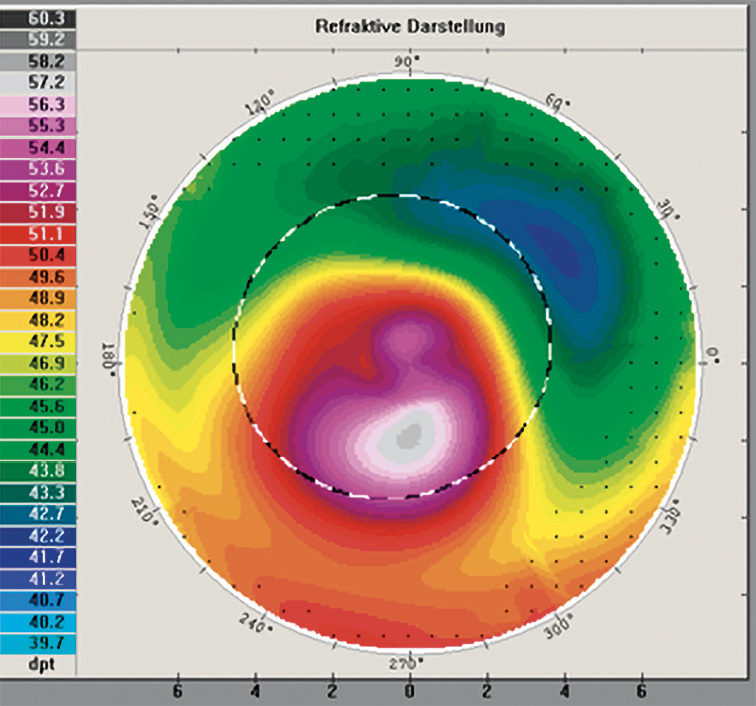
Secondary ectasias
Post-Lasik ectasia is one of the most serious complications after Lasik surgery. The causes that lead to ectasia after Lasik surgery are very different from those of keratoconus. A thin cornea is considered an important risk factor for the development of post-Lasik ectasia. The Lasik procedure consists of creating a flap to correct refractive errors in the anterior part of the posterior stroma by removing tissue. The problem is that the flap does not fuse with the residual stroma.37 The cornea is functionally thinned because the "flap is functionally decoupled from the cornea".25 The most mechanically resistant part of the stroma is the anterior corneal stroma due to the cross-linking of its collagen lamellae. This part of the stroma can no longer contribute to the mechanical stability of the cornea after surgery. The posterior, mechanically weaker part of the stroma is not able to withstand the permanently acting intraocular pressure. The mechanical load capacity of the posterior stroma is half that of the anterior stroma.27 The elastic modulus decreases linearly from the anterior to the posterior stroma, by up to 3 MPa/mm.38 The thicker the flap and the thinner the residual stroma, the more the mechanical resilience of the cornea is compromised. A cornea with an initial thickness of 480 µm and a flap thickness of 150 µm has a functional thickness of only 290 µm when 60 µm of stromal tissue has been removed to correct a myopia of -5 dpt. The intraocular pressure (IOP) acting on the cornea causes a wall tension T in the corneal stroma, which must be neutralised by the collagen fibres. The wall tension depends on the thickness d and the radius r of the cornea and is described by Laplace's (simplified) law as follows σT=(IOP∙r)/2d With an intraocular pressure of 16 mmHg (2128 Pa), a corneal thickness of 530 µm and a radius of 7.8 mm, the wall tension is 118 mmHg (15694 Pa). If only a functional corneal thickness of 290 µm remains after the Lasik treatment, the wall tension increases to a value of 215 mmHg (21797 Pa). With half the mechanical stability of the posterior stroma and almost double the wall tension, the risk of postoperative ectasia is significantly increased.
Diabetes: natural crosslinking
Corneal crosslinking is a procedure for the treatment of primary and secondary ectasia that demonstrates the importance of collagens in corneal structure and stability. Natural crosslinking occurs in diabetes. Clinically relevant changes in the cornea (e.g. decreased corneal sensitivity, increased stiffness) can be detected in more than two-thirds of all diabetics.39 Hyperglycaemia caused by diabetes leads to non-enzymatic protein glycosylation and an associated formation of AGEs (advanced glycosylated endproducts). In diabetics, corneal stiffening is observed as a result of cross-linking of collagens by glycosylation.40,41 Diabetic changes in biomechanics can be detected with air-puff methods. Perez-Rico determined the deformation amplitude with the Corvis ST (Oculus Optikgeräte, Wetzlar). At an HbA1c value of 6%, the amplitude averaged 1.03 mm; at an AbA1c value of 9%, the amplitude dropped to 0.93 mm.41 The lower amplitude of oscillation is a consequence of the stiffening of the cornea.The prevalence of keratoconus is not lower in diabetics than in non-diabetics, but there is a negative association between diabetes and the degree of keratoconus.42 Natural cross-linking in diabetes does not prevent the development of keratoconus, but it does have a beneficial effect on the progression of keratoconus. A protective effect of diabetes on keratoconus is also mentioned by Seiler. However, this protective effect can only be demonstrated for type II diabetes.43 The higher mechanical stiffness of the cornea may also explain why increased intraocular pressure is often measured in diabetics.44 A higher mechanical force is required to applanate a stiffened cornea.
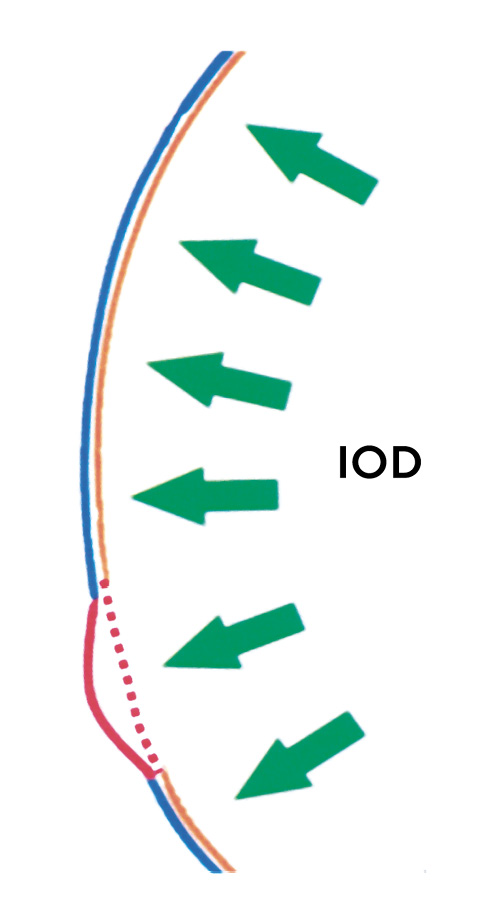
Discussion
For the cornea, the statement "the structure determines the function" applies to a great extent. A constant refraction of the eye, independent of forces (e.g. blinking, intraocular pressure) acting on the cornea, is only given if the cornea can withstand these forces. The biomechanical properties (e.g. tensile strength) of the collagens, which can be derived from their biochemistry, are the prerequisite for the anatomical and functional stability of the cornea. Ectasias of the cornea express themselves in a progressive local or generalised thinning of the cornea, which is accompanied by a steepening of the cornea. They are an expression of a biomechanical weakening of the stroma. Altered biochemical properties of the collagens, which can be caused by collagenases, cause the collagens to slide past each other. An arrangement of the collagen fibres that deviates from the lattice structure additionally leads to a mechanical weakening of the corneal stroma. The cornea is not a linear and homogeneous elastic tissue. Only for low mechanical loads can the cornea be considered a linear material for which Hooke's law can be applied. The elastic modulus of the cornea depends on position, in particular its magnitude decreases from the anterior to the posterior stroma. This is relevant to post-Lasik ectasia because postoperatively the anterior stroma is permanently uncoupled from the residual stroma and can no longer contribute to the mechanical resilience of the cornea. The residual posterior stroma is unable to withstand the deforming effects of intraocular pressure over long periods of time. Diagnosing primary and secondary ectasia is easy thanks to modern imaging and topographic techniques. While imaging techniques such as Scheimpflug photography or optical coherence tomography provide reliable information about the topography, determining the biomechanical properties of the cornea is very difficult. In particular, it is not possible to infer biomechanics from topography. There are no simple measurements that can be related to biomechanics. Even measuring corneal thickness to correct the intraocular pressure reading does not provide reliable information on the mechanical resilience of the cornea. The advantage of using centre thickness as a measure of biomechanics is that this measurement is easy to perform. The use of air-puff methods (e.g. Ocular Response Analyzer, Corvis ST), in which the cornea is indentated with a short air pulse and then the biomechanics of the cornea are inferred from the temporal change in corneal geometry, has led to various new measurement variables (e.g. corneal hysteresis, corneal resistance factor, deformation amplitude). Although these parameters are suitable for clinical practice, they provide only limited information about the actual biomechanical changes that lead to ectasia.
COE Multiple Choice Questionnaire
The publication "Corneal Biomechanics in Keratoconus and Ectatic Corneal Diseases" has been approved as a COE continuing education article by the German Quality Association for Optometric Services (GOL). The deadline to answer the questions is 1st June 2023. Only one answer per question is correct. Successful completion requires answering four of the six questions.
You can take the continuing education exam while logged in.
Users who are not yet logged in can register for ocl-online free of charge here.